Matter and light
Posted by Austin Morris on UTC 2019-07-19 12:22
In a piece written nearly three years ago we cited a remark of the literary critic George Steiner concerning the persistence in our minds of outdated views of the world:
We speak still of 'sunrise' and 'sunset'. We do so as if the Copernican model of the solar system had not replaced, ineradicably, the Ptolemaic.
George Steiner, Real Presences, Faber and Faber, London, 1989, p.3.
Even at this 50 year anniversary of the first moon landing and even at that time when we had humans standing on the moon, so deeply does this Ptolomaic thinking sit in our minds that the title 'Earthrise' was coined for the photograph taken in 1968 depicting the moment when the Earth came into view over the Moon's horizon from a spacecraft in lunar orbit.
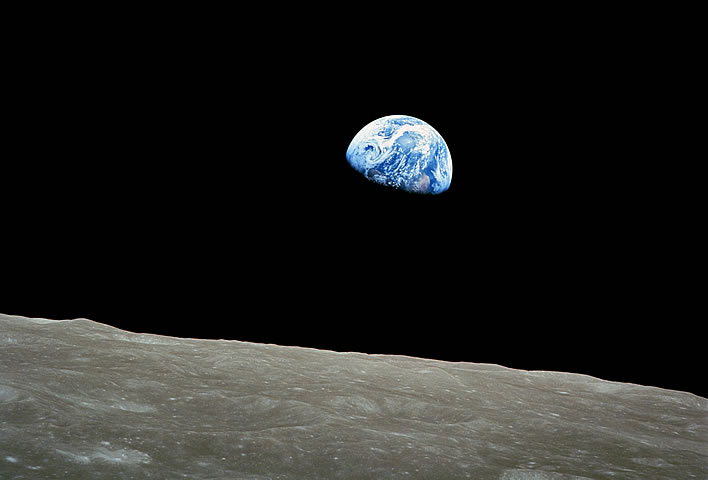
'Earthrise' (slightly cropped in the vertical). Image original: NASA.
Consider that viewpoint for a moment: here is an image from a camera in a spacecraft orbiting the Moon, which in turn is orbiting the Earth, which in turn is orbiting the Sun. It is even possible in a different reference frame to see the Moon as orbiting the Sun, not the Earth – the two in 'companion orbits' around the Sun. From all other reference frames than that of the camera the image shows the camera, the viewer and the spacecraft 'descending' relative to the Earth, not the Earth 'rising'. In order to conform to our Ptolemaic imaginations the image itself was then rotated by 90° to make the Moon's surface 'down' and the Earth 'up'.
Picking up on this theme, a long essay could be written on the number of expressions in English which rely on pre-relativistic, even pre-Newtonian views of the world. The counter-intuitive obscurities of the atomic-scale world have barely had any effect on the way we talk about matter and energy. We humans understand the world around us in ways that often remain conceptually rooted in the Middle Ages.
Let's take an example. Here is a photograph of a block of adhesive marker strips. What colour is it?
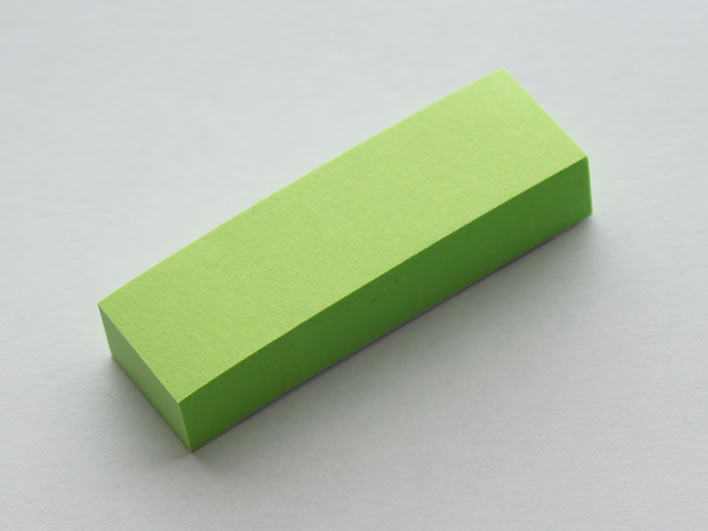
Apple green?
The light that reaches our eyes from it (and from the photo of it and from your screen) has an electromagnetic spectrum that corresponds to a colour we might call apple green – after it has passed into the receptors of our eyes and they and our brain cells have done their magic.
But that's not the colour of the strips themselves. It's just the spectrum of the light that is scattered from the surface of the paper. All the other wavelengths of the other colours are absorbed and not returned.
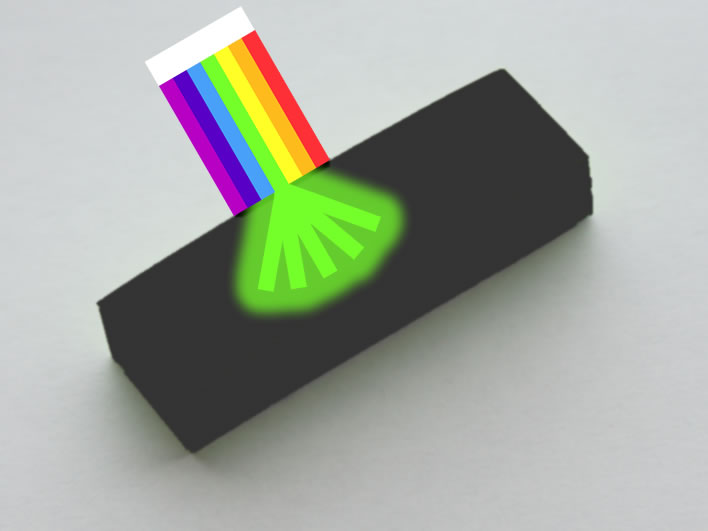
If we want to be scientifically accurate, we should say that the strips have an absorption spectrum consisting of all the wavelengths that are not incuded in the spectrum of apple green: all these non-apple-green wavelengths from the light falling on the strips are absorbed.
Conversely, we might call the light coming from the strips the scattering spectrum. The wavelengths that make up the colour apple green are scattered at the surface, hence this is the colour of light we see when we look at the strips.
But that, as we have noted, is not the colour of the strips, just a property of their surface. That property in turn depends on the properties of the atoms and molecules on (and close to) the surface of strips. These molecules will absorb the light falling on them, except for the apple-green wavelengths, which they will scatter. The scattering may emit light in all directions more or less equally or preferentially in certain directions. The object only has colour when the right sort of light falls on it. And that colour is expressed in the light, not the object itself.
Put another way, if a particle of matter has a predeliction for an incoming photon of a particular wavelength, it will capture it and send it packing again in some other direction. Any other photon will be either captured and absorbed or allowed to pass.
The scattered incident light is not only the source of the colour we see in an object but also a factor in its intensity. For example, the principal reason that alpine flowers display such intense colouring is nothing to do with any property of the flowers themselves but is rather everything to do with the intensity of the high-altitude light that falls on them:
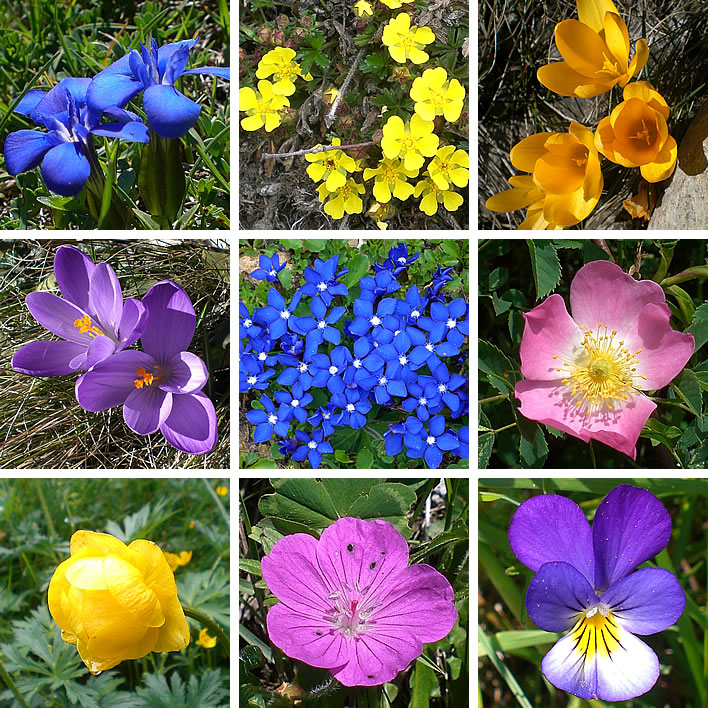
NB: All these images were taken at altitudes between 1,300 and 2,000 metres above sea level. These images have not been filtered in any way to enhance the intensity of the colours. Whatever deviations from the 'real' view there are, are the artefacts of the conventional processing of digital images and of viewing them on light-radiating screens with varying gamma curves (the images will be brighter for Mac users, for example). That these artefacts are minimal can be seen from the dampened colours of the backgrounds. The UV-rich light at high altitudes also increases the colour intensity of the flowers, making them more attractive for the visual spectrum of polliinating insects. Image: FoS.
If we were to illuminate the block of strips with light which had the apple-green wavelengths filtered out, the strips would appear to be some shade of black, depending how good our filtering was. There is no apple green in them at all. With perfect filtering of all the wavelengths emitted from the strips we would see something like this:
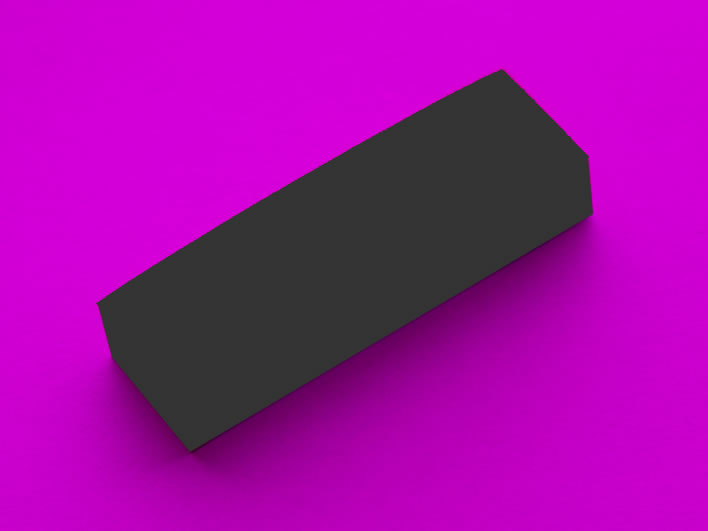
If the block of strips is not apple green, what colour is it?
It is therefore not correct to speak of the colour of the block of marker strips as being apple green: the colour we see depends on the spectrum of the light falling upon it. In fact, broadly speaking, the surface of the block of strips does not have any colour of its own at all – even the molecules of dye that create the green colour are not themselves green.
The only colour they have comes from the colour spectrum of the electromagnetic radiation that they are emitting in accordance with their temperature, and that is certainly not green.
At room temperature this would be a long infrared wavelength that we cannot see. This is arguably the true colour of the strips – and the tabletop, too. So that if we exclude all incident light – that is, we are in a room without windows and lighting – this is what we would see:
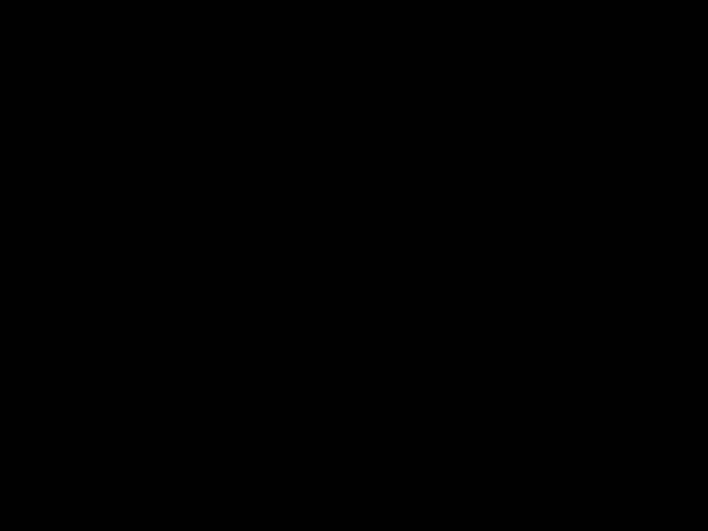
We might call that the 'intrinsic colour' of the block of marker strips.
The strips will have slightly different radiative characteristics from the table they are on. If they are both at the same temperature that difference will be minute, meaning that, in effect, we will not be able to make out the strips on the table – and not be able to see the table either without some special equipment. Even then we will only be able to make out distinct objects where there are temperature differences.
Putting it another way
Once we have removed all the colour artefacts caused by the scattering of incident light, we enter a world where the colour of an object depends largely upon its temperature.
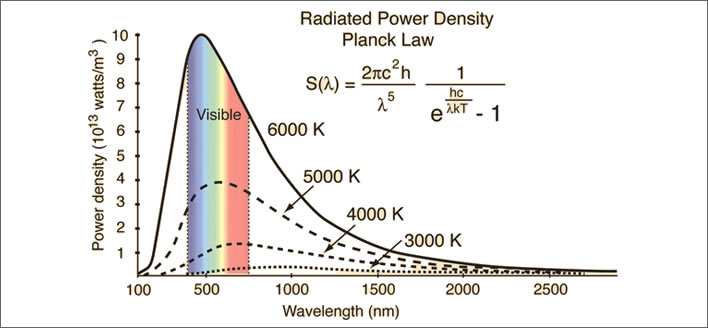
Emissions of electromagnetic radiation at the surface of the Sun, temperature 6,000°K (5,727°C). Hugely energetic emissions with a lot of blue, ultraviolet light and wavelengths outside the visible spectrum. As the temperature reduces the intensity of the output power reduces and the principal wavelength region shifts from blue to red and into the infrared. The output power curves for 5,000°K, 4,000°K and 3,000°K are also shown. NB: 1) the Sun is not a perfect blackbody and 2) this is not the solar spectrum that we on Earth see: the passage through atmosphere changes the curve. Superimposing a rainbow spectrum on the curve is potentially misleading: the light spectrum emitted at a particular temperature is a mixture of all the wavelengths under the curve in proportion to their intensities. Clear? Of course! Image: Hyperphysics.
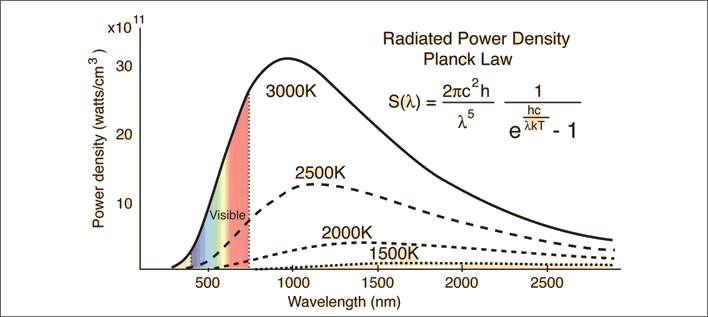
Emissions of electromagnetic radiation at 3,000°K (2,727°C). The 3,000°K curve from the previous picture plotted on a rescaled y-axis. As the temperature reduces the intensity of the emissions reduce and their wavelengths extend. The output power curves for 2,500°K, 2,000°K and 1,500°K are also shown. By 1,500°K (1,227°C) the power output is dramatically reduced and the wavelength has increased considerably. Image: Hyperphysics.
We could take the 'intrinsic colour' of an object to be the spectrum of light emitted on account of its temperature. If we could heat up our paper strips to poker heat they would glow reddish orange, much like the iron of the poker. Well, not quite – as an object emits electromagnetic radiation, the atoms and molecules of the object emit their own particular spectra, which can be used as a kind of fingerprint in their identification. This uniqueness is why we can speak of intrinsic colour in respect of emission spectra. But these spectra we usually only see at high temperatures, well outside the range of normal life.
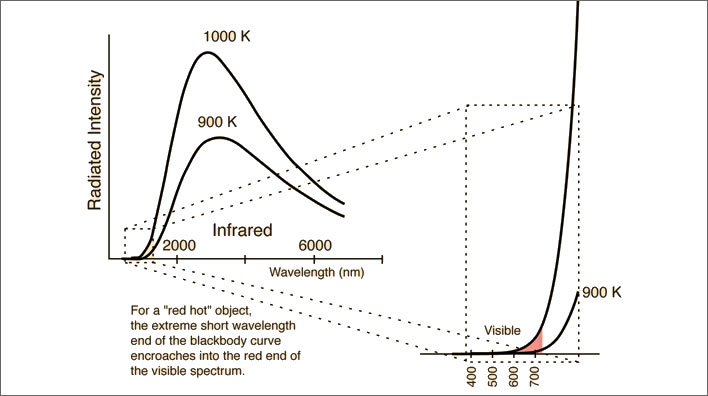
Emissions of electromagnetic radiation at 1,000°K (727°C), around the temperature of a glowing hot poker. A portion of the curve has been enlarged to show the small proportion of red light emitted. At 1,000°K the poker will be glowing distinctly; at 900°K the glow will be barely visible. Our eyes are not our only sensors: our skin will easily detect the infrared emissions if we put a hand near the poker. Image: Hyperphysics.
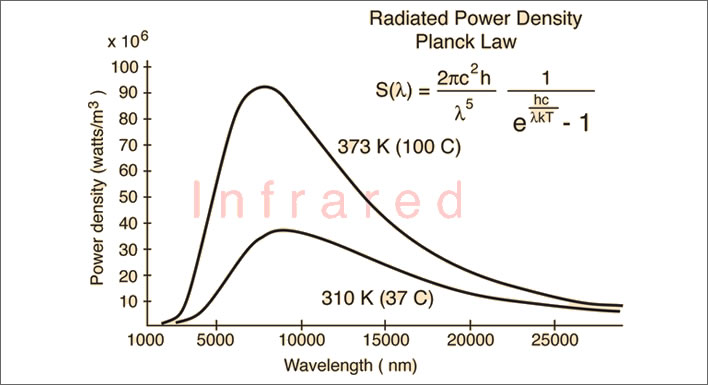
Reducing the temperature range to that of normal life, there are now only weak emissions and only invisible infrared. The temperature sensors in our hand will be able to detect the heat when the hand is held close to a boiling kettle at 373°K (100°C). At the temperature of the human body 310°K (37°C) the emissions are extremely weak and stretch very far into the infrared. NB: the x-axis is 10x longer than that in the previous diagram. A temperature of 20°C (not plotted here) would be even flatter and peak at 10,000 at just under 9 on the y-axis. Under normal circumstances our hands cannot sense this heat at a distance because they are also at body temperature. Thermographic cameras can detect wavelengths up to around 15,000 nm with great sensitivity. Image: Hyperphysics.
Without incident light, objects at the same temperature will appear to be the same colour – hence the background grey of thermal imaging cameras. Because of the high-sensitivity of these cameras they can differentiate between very small temperature differences and display these differences in false colours. At everyday temperatures objects do not emit visible light and so can be said to have no colour – which is the same as saying that they are black.
Another example:
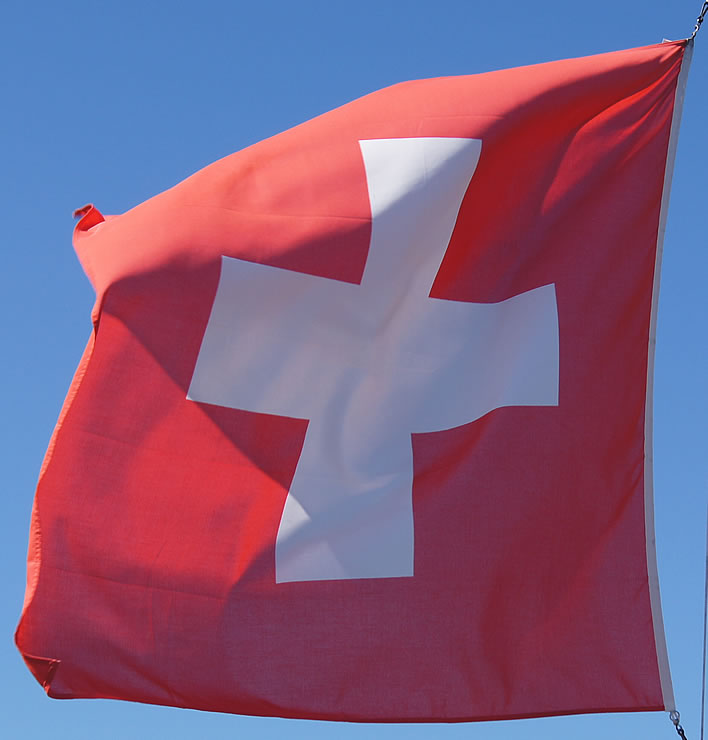
The colour of the background of the Swiss flag is not red – it is the same intrinsic colour as the white cross in the foreground (since we can assume that all the flag is of the same material and at the same temperature). In the real world the colour of the Swiss flag is a monocolour – that is, black – at normal temperatures. If we look at the flag without any incident light, this is what we see:
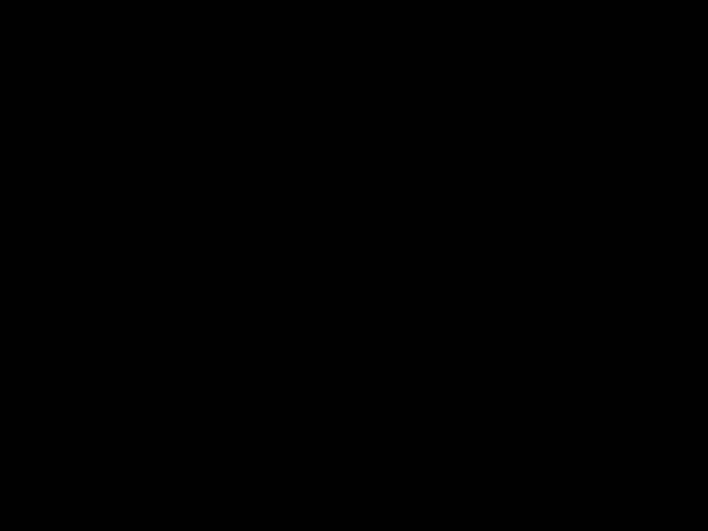
It just happens that the molecules on the red part scatter red light and absorb everything else and the molecules of the white part scatter a range of visible wavelengths almost equally. Out of this mixture of wavelengths our eyes and brain cause us to see some form of white colour.
Note that, just like the block of marker strips, the flag does not have colours which only become visible when light falls upon it – the light falling upon it creates the colours. We do not go into a dark room, switch the light on and see our red flag now illuminated. When it was not illuminated, it was not red or white. It becomes red as a result of the illumination.
We material girls and boys live in a world of matter which, at normal, human temperatures is invisible and without perceptible colour. The infrared/microwave radiation it emits is invisible to us without technical aids. We see light, not matter. Only light has colour. Remember that the next time you want to paint the living room and change the diffuse refraction characteristics of its walls. Language matters.
The world in which we live therefore consists of two great domains: the domain of matter and the domain of electromagnetic radiation (which in the end may even be one and the same thing). Our existence is to be found in the interactions between the two.
0 Comments UTC Loaded:
Input rules for comments: No HTML, no images. Comments can be nested to a depth of eight. Surround a long quotation with curly braces: {blockquote}. Well-formed URLs will be rendered as links automatically. Do not click on links unless you are confident that they are safe. You have been warned!